Colour is a perceptual interaction arising from our ability to discriminate between different wavelengths of light from within a narrow band of electromagnetic radiation. Light itself has no colour. The colour of a specific wavelength can change according to context. For example, in the above picture, despite having the same spectral reflectance, the ‘X’ appears to be different when set against the two different backgrounds (Albers J. 1975).
In his book, The Symbolic Species, Terrence Deacon talks about the co-evolution of language and the brain. He makes reference to the exciting cross-cultural linguistic research of Berlin and Kay. Berlin and Kay showed that the basic colour mechanisms were consistent across different cultural groups and were based on the underlying psychophysical similarities of the human brain.
“In summary, the universality of colour term reference is an expression of shared neurological biases, but—and this is a crucial point—the translation of this biological constraint into a social universal is brought about through the action of nongenetic evolutionary forces.”
(Deacon, (1997), The Symbolic Species, p119-120)
Language requires the coordination of perceptually grounded categories with a socially-negotiated set of shared linguistic conventions to express them; i.e. language is based on shared groups of meanings that arise from our perceptual interaction with the external world and the way in which we convey that relationship to other human beings. Deacon’s opinion is that neurological predispositions and socio-ecological constraints sponsored the development and evolution of language, and that the subsequent feedback system gave rise to a complex coevolution of the two. Founded neurological determinism within evolutionary and socio-ecological boundaries drives the core of his argument.
There is one fundamental aspect of the conscious experience Deacon doesn’t present. The way in which humans interact with our environment is largely determined by how we ‘perceive’ that environment. What we perceive though, is not always what the rods and cones of our retina detect.
The colours to which we are perceptually attuned do not correlate with the wavelengths of light that selectively, but not exclusively, stimulate our rods and cones and subsequently the retinal ganglion cells. There are two chromatically opponent cardinal mechanisms that correspond to the properties of the receptors located in the retina and the Lateral Geniculate Nucleus. These wavelengths roughly equate to reddish, greenish, yellowish and bluish as shown in the following picture:
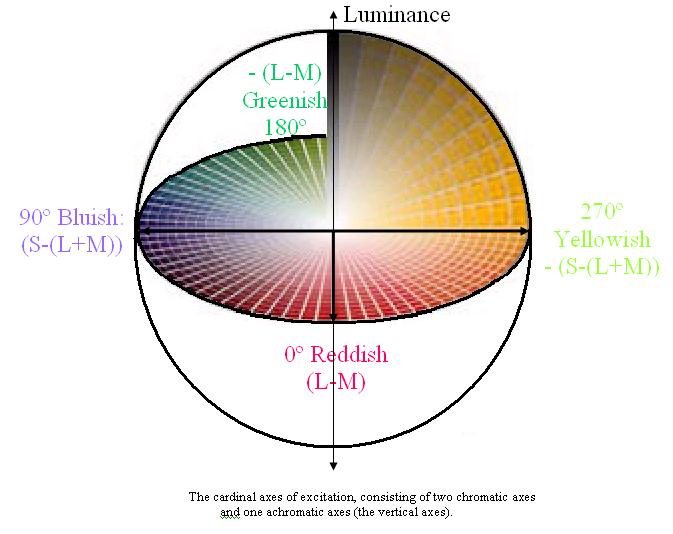
Whilst the cardinal axes are defined on the basis of chromatic contrast detection (Krauskopf, Williams, Heeley 1982), a second set of axes are used to describe colour identification (Boynton 1965). These are the unique hues (Hurvich, Jameson 1957). The unique hue axes differ from the cardinal axes in that they are defined based upon the perception of colour. The identification of the perceptual colour axes, i.e. the unique hues, is based on both experimental studies (Hurvich & Jameson, 1956; Boynton et al., 1964) and cross-cultural linguistic evidence (e.g. Berlin & Kay 1969; Heider & Olivier, 1972). The four unique hue axes do not lie along the poles of the four cardinal axes (Webster, Miyahara, Malkoc & Raker 2000), which demonstrates the discrepancy between the reception and perception of colour.
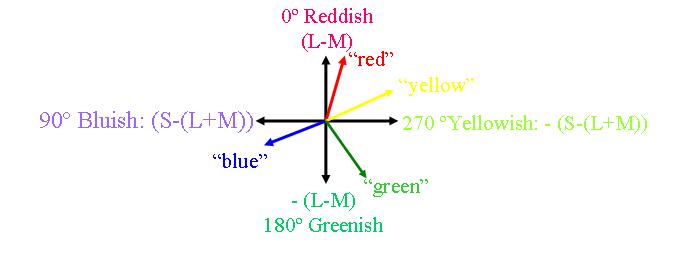
The unique hues are red, yellow, green and blue and their relations of hue similarity have an opponent structure. They are colours that cannot be made up of a combination of other colours. However, other colours can readily be described as mixtures of the unique hues. For example, the binary hues are perceptual mixtures of the unique hues, they include purple (blue and red), orange (red and yellow), olive (yellow and green) and turquoise (green and blue). It is important to make clear that hue mechanisms do not correspond to the spectrally opponent LGN cells because these cells respond not only to chromatic variations but also to achromatic stimuli and to spatial patterns.
Allow me to describe this discrepancy another way:
Colour is in some ways a result of ratios of activation. The experience of colour is dependent on the existence of two or more cones with different spectral sensitivities. In humans there are normally three different types of cone-cell used to sample the continuous spectrum of light. Each of the cones absorbs a range of wavelengths but preferentially absorbs photons at specific wavelengths.
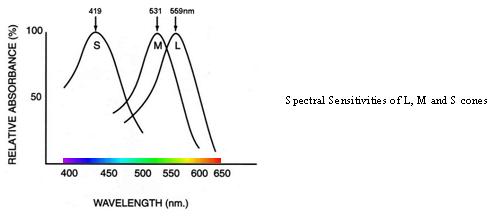
The L-cones are most likely to absorb wavelengths at around 559nm, the M-cones at around 531nm and the S-cones at around 419nm (Dartnall et al. 1983). Each cone type contains a different photopigment and each photopigment has a distinctive wavelength where it is most likely to react. At the wavelengths outlined above, the probability that a photon is absorbed increases and a greater proportion of photopigment is bleached. Cone sensitivity at any wavelength is dependent on the light intensity, and absorption of a photon always results in the same electrical response regardless of the stimulus wavelength. In essence, the wavelength is lost at the photoreceptor i.e. specific information about wavelength is converted to much less precise information coded by the photoreceptors.
Wavelengths are turned into a specific pattern of signals and colour appearance is influenced by the ratios of cone excitations and by the overall levels of cone excitation caused by the prevailing illumination. There is significant overlap in the response curve to spectral sensitivities of the three cone types resulting in a relatively high correlation and redundancy of signals (Lennie, 2000). This redundancy is filtered later on in the system and higher order chromatic mechanisms are required to account for the unique hues (red, green, blue and yellow) of colour perception.
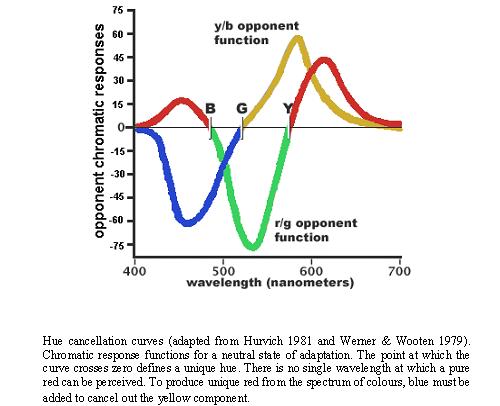
When average subjects are asked to mix four specific wavelengths of light (corresponding to the cardinal colours), using a hue cancellation procedure, to exactly match (or cancel to gray) the color of any single wavelength, their average mixtures follow the hue cancellation curves shown above (Werner & Wooten 1979). It is important to note that the mixing of opponent curves is neither as symmetrical nor evenly spaced across the spectrum as the cardinal colour axes would predict. This discrepancy suggests that the subjective sense of uniqueness or purity of hues for highly specified wavelengths is not determined by the retinal or LGN opponent-process cells. The LGN opponent cells are responsive to chartreuse/violet and teal/cherry i.e. greenish-yellow/deep-purple and greenish-blue/bright-red (Abramov & Gordon 1994:468).
Psychophysicists have known for a long time about the many discrepancies between accepted opponent-colours theory and the empirical phenomena (Judd 1951). An analogue of this discrepancy is the apparent mismatch that exists between the Cardinal axes (Krauskopf, Williams, Heeley 1982) and the Unique Hue axes (Hurvich, Jameson 1957). Whilst the cardinal space can be directly related to cone (three-colour) excitation, the Unique Hue space cannot. Thus, the chromatic cardinal mechanisms cannot be considered as correlates of the perceptual unique hues (Valberg 2001).
If you’ve followed me up to here, then you have done a great job! If you haven’t, then perhaps I need your help to find out how I can express myself better!!!
So, after this discussion about how the discrepancy between wavelength reception and colour perception, we come to the question, are colours in the brain?
To answer this question, I will refer to a phenomenon in the environment, metamers, and a phenomenon found in some humans, synaesthesia.
Metamers are objects with distinct spectral reflectance properties that nonetheless appear the same. Their apparent similarity occurs because the colour of an object depends on how wavelengths reflected from that object activate each of three types of photoreceptor in the eye (Rosenthal 2001). The brain computes visual colours by analysing the relative excitations of these photoreceptors. Thus, colours result not from specific wavelength, but from ratios of activation. Hence, humans see many combinations of wavelength as the same colour, because many combinations will produce the same ratio of activation of the three types of cone cell. So, in some philosophical circles, colour could be seen as something in your brain.
It is the phenomenon of synaesthesia in colour-blind people which is perhaps most intriguing for our question. Ramachandran & Hubbard have described a case of a colour-blind man with synaesthesia. Being colour blind, there are certain colours he has never seen. However, his synaesthesia sometimes elicits extrasensory colours, or “Martian Colours” as this synaesthete calls them. Even in regular synaesthetes, there are reports of colours being elicited through their synaesthesia that confer unusual tints to the colours they see in the natural world. Could this finding suggest that colour is in the brain?
I have to say that, for the moment, I shall sit in the interactionist camp on this one!!!